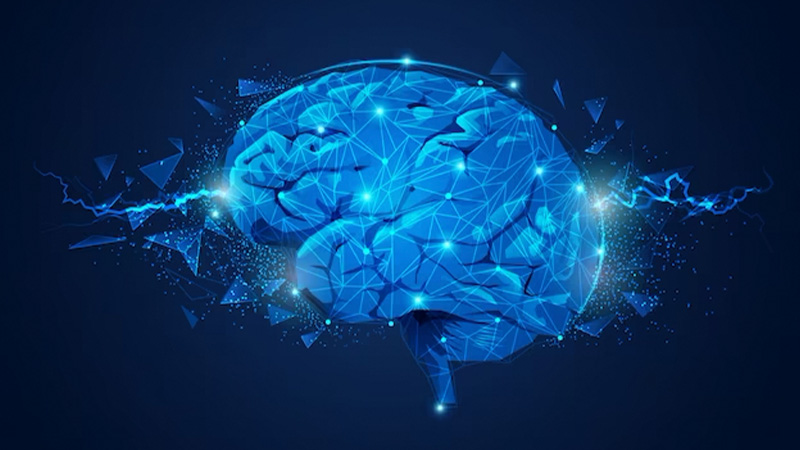
RESEARCH ARTICLE
Use of either transcranial or whole-body photobiomodulation treatments improves COVID-19 brain fog
Abstract
There is increasing recognition of post-COVID-19 sequelae involving chronic fatigue and brain fog, for which photobiomodulation (PBM) therapy has been utilized. This open-label, pilot, human clinical study examined the efficacy of two PBM devices, for example, a helmet (1070 nm) for transcranial (tPBM) and a light bed (660 and 850 nm) for whole body (wbPBM), over a 4-week period, with 12 treatments for two separate groups (n = 7 per group). Subjects were evaluated with a neuropsychological test battery, including the Montreal Cognitive Assessment (MoCA), the digit symbol substitution test (DSST), the trail-making tests A and B, the physical reaction time (PRT), and a quantitative electroencephalography system (WAVi), both pre- and post- the treatment series. Each device for PBM delivery was associated with significant improvements in cognitive tests (p < 0.05 and beyond). Changes in WAVi supported the findings. This study outlines the benefits of utilizing PBM therapy (transcranial or whole-body) to help treat long-COVID brain fog.
1. INTRODUCTION
COVID-19 has had a devastating impact on global public health. However, the major brunt of this pandemic has affected patients with underlying chronic disease and other morbidities (obesity, diabetes, etc.), secondary to the cytokine storm and acute respiratory distress syndrome (ARDS) [1, 2]. COVID-19 has been managed with vaccinations, monoclonal antibodies, immunomodulators, and antiviral agents. However, the global population is now dealing with the major effects of post-pandemic complications termed “long COVID” [3–5]. Two specific symptoms that stand out are general fatigue and cognitive dysfunction, also termed “brain fog” [6–8]. There are no directed therapeutics for this complication developed to date.
Given the relatively high sensitivity of the coronavirus to physicochemical modalities, several forms of light treatment have been attempted. A popular approach is using ultraviolet ionizing radiation extrinsically or a combination of dye and light for systemic use, termed photodynamic therapy (PDT) [9–11]. In contrast, biophotonics treatments directed at shoring up the antimicrobial host immune response and reducing the inflammatory cytokine storm damage are termed photobiomodulation (PBM) therapy [12–14]. A rationalized combination of the two treatments is conceivable but requires careful application [15]. The presence of multisystem dysfunctions noted with COVID-19 has raised significant interest in both targeted (e.g., transcranial) and transdermal, whole-body treatments and how these treatments can be effectively employed [12, 14, 16–19]. There has been tremendous progress in our understanding of the photobiological mechanisms of PBM [20]. Three discrete sites of interactions, namely the mitochondrial cytochrome C oxidase, cell membrane receptors and transporters, and the extracellular activation of a growth factor, TGF-β. These mechanisms appear to be cell- and tissue response-specific and may often be concurrently involved.
There have been some recent publications outlining the evidence for the use of PBM treatments for acute COVID-19 complications [17, 21–31]. The use of PBM in COVID-19 management was prompted by its efficacy in relieving ARDS [32–36]. PBM treatments have focused on mitigating acute pulmonary inflammatory complications that have been supported by several controlled lab and animal studies [37–39]. The use of multiple inflammatory stimuli in these studies is particularly worth emphasizing, as this suggests that PBM appears to target the underlying pathophysiological process. Although severe acute-phase SARS-CoV-2 infections are not known to directly cause neurological damage, the long-term effects of systemic inflammation and vascular dysfunction could indirectly contribute to neurological damage. The role of the ACE2 receptor in mediating viral attachment in a wide range of cells in the cardiovascular and immune systems has been noted [40, 41]. The ACE2/Ang [1–9] receptor is essential in maintaining blood pressure and vasodilation. With infection, the virus binds ACE2 and disables this protective mechanism, which can result in a cytokine storm, coagulation, increased vascular permeability, and acute lung injury [42–44]. Persons with pre-existing ACE2 deficiencies, such as those with diabetes or hypertension, are at even greater risk. In the brain, loss of ACE2 impairs autoregulation of blood pressure and endothelial cell function [45]. Cognitive alterations have been observed in patients who have recovered from the acute phase of COVID-19. These include concentration memory, executive function, information processing, and language [46].
A major question in the field has been the delivery of PBM doses to precise anatomical sites for optimal efficacy. There have been elegant, controlled animal studies with the MPTP (1-methyl-4-phenyl-1,2,3,6-tetrahydropyridine)-induced Parkinson’s disease model as well as the myelin oligodendrocyte glycoprotein (MOG)-induced experimental autoimmune encephalitis (EAE) model of multiple sclerosis [47, 48]. These groups utilized whole-body systemic PBM treatments and observed reduced clinical symptoms, biochemical, molecular, and histological changes, demonstrating therapeutic efficacy. Strikingly, the MPTP-induced PD model in rodents and primates demonstrated equivalent efficacy when either systemic whole-body or transcranial PBM treatments were performed [49]. In contrast, studies on depression, Alzheimer’s, and traumatic brain injury have utilized transcranial treatments with PBM helmets or headsets [50–52]. The striking clinical efficacy of PBM treatments in supportive cancer care has also highlighted the equivalence of intraoral and extraoral treatments [53–55]. The growing interest in extending PBM treatments to the broader supportive cancer care complications, such as tissue fibrosis, malaise, fatigue, cancer cachexia, and cancer brain has led to practical clinical considerations of systemic, multiorgan treatments for optimal benefit. These treatments have now become clinically viable with large light (LED) panels and beds that are cost-effective for both providers and patients.
Hence, this pilot study was aimed at examining the effectiveness of a PBM bed and helmet in relieving long COVID symptoms. As a proof of principle study, the design used only active treatment. Neuropsychological/cognitive and quantitative electroencephalogram (EEG) assessments were performed. The PBM treatments were delivered three times a week for 4 weeks using either a helmet for transcranial (tPBM) or a light bed for whole-body (wbPBM) treatments.
2 MATERIALS AND METHODS
Participants and clinical study design
Subjects were recruited through word of mouth and social media (Facebook) announcements. All subjects had a positive PCR test for COVID-19 and had recovered from the acute phase of infection. Inclusion criteria were the following: They presented with cognitive decline (brain fog) of at least 5 months duration with minimal or no improvement. Subjects described their symptoms with statements, such as: poor articulation, lack of recall, especially numbers, slow reactions, forgetting names and directions, clumsy, easily confused, losing train of thought, mentally overwhelmed, and mentally fatigued. Exclusion criteria were the following: Subjects were under the age of 18, prisoners, military recruits, persons with other disorders that might result in cognitive impairment, persons not competent to give informed consent, and other vulnerable persons. There were two separate groups, n = 7 each (digital randomizer program). Group 1 consisted of those receiving tPBM; and Group 2 consisted of those receiving wbPBM. A total of 16 subjects were initially recruited for the study, but two failed to complete it. The study design was approved by the Institutional Review Board (QuietMind Foundation #06092022). All subjects signed the approved informed consent, and procedures were followed in accordance with the Declaration of Helsinki.
Cognitive assessments
Subjects were evaluated using the Montreal Cognitive Assessment (MoCA), digit symbol substitution test (DSST), trail-making tests A and B, and physical reaction time (PRT) at visits 1, 6, and 12 before and after PBM treatments. The MoCA is a 30-item test that assesses language, memory, visual and spatial thinking, reasoning, and orientation skills. It is thought to be more useful for mild cognitive impairment than the mini-mental status exam, and normal subjects usually score 25 to 30 [56]. The digit symbol substitution test (DSST) assesses cognitive impairment [57]. Nine digit-symbol pairs are listed at the top of a page, followed by a list of digits. Under each of the digits, the subject records the corresponding symbol. The number of correct answers in 90 s is recorded. A higher score is better. Trail-making tests are useful for assessing visual attention and task switching [58]. The Trails A test consists of only numbers, where each number is inside a circle randomly arranged on a page. The task is to draw a line to connect the correct number/circle in ascending order as quickly as possible. The Trails B test consists of numbers and letters, each inside a circle randomly placed on the page. The task is to draw a line, switching between connecting a number and then connecting a letter, in ascending order of each, as quickly as possible. There are 25 circles. Shorter test completion times indicate improved cognitive performance. The PRT test consists of the time to respond to an auditory signal on computer-based software.
Cognitive assessments during electroencephalography
The EEG was recorded using the WAVi system (WAVi Boulder, CO, USA) sampled at 250hz and bandpass filtered between 0.5 and 30hz. Electrodes were placed according to the 10–20 system using a cap with 19 electrodes and tworeference electrodes on the earlobes. Electrode impedances below 30 ohms were established prior to testing. The P300 event-related potential measures the stimulus-evoked subject response with the EEG, which is best assessed over the parietal lobe. The time to response (lower P300T) and amplitude (voltage) of current (higher P300V) reflect improved cognitive function in this test. The Eriksen Flanker tasks examine the ability of the subject to suppress inappropriate responses in a particular context representing cognitive processing in the presence of distracting information (noise). The target is flanked by non-target stimuli corresponding to either the same direction as the target (congruent flankers), the opposite response (incongruent flankers), or neither (neutral flankers). This assessment is focused on the anterior cingulate cortex (ACC) in the frontal lobe, which is responsible for a wide variety of autonomic functions. The time to response (lower Flanker T) and amplitude (voltage) of the current (higher Flanker V) reflect improved cognitive functions.
Photobiomodulation (PBM) treatment schedule and equipment
Participants received three treatments a week (48 h between treatments) for 4 weeks. Group 1 received tPBM treatments; Group 2, wbPBM treatments. The tPBM treatments were administered with the Neuroradient 1070 light-emitting diodes (LED) helmet (Neuronic Devices Ltd, Ireland). This helmet is lined with LEDs that emit photons at a wavelength of 1070 nm (100% duty cycle, CW) with a tissue surface irradiance (power density) of 24 mW/cm2 for 14 min for a fluence of 20.2 J/cm2, photon fluence of 24.2 p.J/cm2 and 5.4 Einstein [59]. The wbPBM treatments were performed with the NovoTHOR (THOR photomedicine, London, UK) light bed that has 660 nm and 850 nm LEDs (100% duty cycle, CW) at the treatment surface irradiance of 24 mW/cm2 for 14 min for a fluence of 20.2 J/cm2, photon fluence of 34.3 p.J/cm2 and 769 Einstein [60–62]. Based on the reported surface areas of the scalp (650 cm2) and total body (18 000 cm2) the bed has a 27 (cumulative fluence) to 39 (cumulative photon fluence) higher dose than the helmet. As a proof of principle study, the design used only active treatment. Subjects were randomly allocated to receive either PBM treatment using a digital randomizer program. Treatments were given thrice a week for four consecutive weeks using either a helmet for transcranial (tPBM) or a light bed for whole-body (wbPBM) treatments (Figure 1A,B).
Statistical analysis
Data was organized in Excel (Microsoft) and analyzed using Pearson’s linear regression for correlation and a two-tailed, paired T-test for pre- and post-treatment comparison using GraphPad Prism (v9.0.0, GraphPad Inc., San Diego, CA).
3 RESULTS
Study demographics
Of the 16 subjects initially recruited for the study, one subject failed to report for testing and treatment. Another subject withdrew from the study after a single session due to an inability to participate in repeated treatments and evaluations schedule. Fourteen subjects completed all evaluations and treatments, 10 females and four males with ages ranging from 37 to 42 years, with a median age of 56 years, and an equal number (7 each) received either PBM treatment (Figure 1C). All subjects reported improvements in their symptoms, and no adverse events (anticipated or unanticipated) were encountered during the course of the study.
Overall treatment period and outcomes assessments
All outcomes from pre- and post-PBM treatments at 1, 6, and 12 days were collected and analyzed that did not show any significant difference. This indicates PBM treatments do not interfere with the assessments performed and that any benefits require longer-term treatments. Further data analysis of the pre-1st day and post-6th day did not show significant differences, indicating this treatment period is also insufficient for therapeutic benefits. The remaining results section focuses on the pre-PBM treatment on Day 1 versus the post-PBM treatment on Day 12 where we observed maximal therapeutic changes that demonstrated statistical significance.
Neuropsychological cognitive assessments
Results for the paired t-test comparisons, pre- versus post-testing after 12 treatments, for each cognitive test for Group 1 (tPBM), and for Group 2 (wbPMB) are presented separately in Figure 2A,B. For Group 1, each cognitive test showed significant improvement (p < 0.05 or beyond), except for PRT. For Group 2, each cognitive test also showed significant improvement (p < 0.05 or beyond), except for TrA and PRT. The individual results are discussed below.
FIGURE 2
Assessing the cognitive status pre-PBM treatment (Day 1) versus post-PBM treatments (Day 12), we noted significant improvements in MoCA scores following tPBM (27 ± 1.53 to 29.42 ± 0.97, n = 7, p = 0.052), and wbPBM (25 ± 3.21 to 29.14 ± 1.21, n = 7, p = 0.052) (Figure 2C). This improvement was not affected by gender (Female 3.5 ± 2.5, n = 10 versus Male 3.2 ± 2.6, n = 4, p = 0.88). In contrast, younger subjects below 65 years (4.6 ± 2.3, n = 9, p < 0.005) appear to benefit more significantly from PBM treatments compared to subjects above 65 years (1.4 ± 1.1, n = 5). However, this could be ascribed to unequal recruitment numbers in this study, which needs to be investigated further.
3.3.2 Digital symbol substitution test
This test combines visual neurocognitive perception, processing, and digital execution. Assessments of subjects at pre-PBM treatment (Day 1) versus post-PBM treatment (Day 12) noted significant successful completion scores following tPBM (50.28 ± 8.34 to 64.71 ± 4.11, n = 7, p = 0.0028), and wbPBM (42.14 ± 13.56 to 57.57 ± 10.47, n = 7, p = 0.036) (Figure 2A,B,D). This test did not demonstrate any statistically significant difference among gender (Female 14.4 ± 8.1, n = 10 versus Male 18 ± 12, n = 4, p = 0.61) or age (< 65 years 16 ± 10.3, n = 9 versus >65 years 14.4 ± 7.3, n = 5, p = 0.74).
3.3.3 Trail A and B test
This test also assesses the combination of visual neurocognitive perception, processing, and digital execution. Assessments of subjects at pre-PBM treatment (Day 1) versus post-PBM treatment (Day 12) noted a reduction in time to successful completion of Trail A following tPBM (65.43 ± 14.59 to 43.43 ± 7.11, n = 7, p = 0.0062), and wbPBM (60.29 ± 27.09 to 52 ± 16.93, n = 7, p = 0.508) (Figure 2A,B,E). Although the wbPBM demonstrated a reduced test completion time, it was not statistically significant. Similarly, the Trail B test analysis demonstrated reduced completion times following tPBM (111.86 ± 50.67 to 58.57 ± 13.07, n = 7, p = 0.0318), and wbPBM (102.43 ± 39.16 to 64.14 ± 14.25, n = 7, p = 0.043) (Figure 2A,B,F). This test also did not demonstrate any statistically significant difference for either Trail A or B test scores among gender (Female −11.7 ± 9.9 s and −36.1 ± 44.7 s, n = 10 versus Male −31 ± 18.4 s and −70.2 ± 26.6 s, n = 4, p = 0.12 and 0.11 paired T-test respectively), age (<65 years −16.3 ± 15.4 s and −45.8 ± 50 s, n = 9 versus >65 years −18.8 ± 16 s and −46 ± 29.1 s, n = 5, p = 0.79 and 0.99 paired T-test, respectively).
3.3.4 Physical reaction time
This test assesses the combination of auditory neurocognitive perception and processing, followed by digital execution. Assessments of subjects at pre-PBM treatment (Day 1) versus PBM post-treatments (Day 12) noted a reduction in response time following tPBM (0.36 ± 0.12 to 0.26 ± 0.03, n = 7, p = 0.069), and wbPBM (0.36 ± 0.05 to 0.32 ± 0.07, n = 7, p = 0.23) (Figure 2A,B,G). Although the data from all three groups that noted reduced reaction times showed statistical significance, neither tPBM nor wbPBM individually noted any statistical significance. The trend for improved response times did not appear to be affected by age (< 65 years −0.08 ± 0.1 s, n = 9 versus >65 years −0.05 ± 0.5 s, n = 5, p = 0.56). However, a statistically significant difference was noted with gender (Female −0.92 ± 0.1 s, n = 10 versus Male −0.02 ± 0.01 s, n = 4, p = 0.04 paired T-test). However, the increased improvement in females could be ascribed to the increased subject numbers (n = 10 vs. 4) that need to be investigated more carefully.
Quantitative EEG assessments
Results for the paired t-test comparisons, pre- versus post-testing after 12 treatments, for quantitative EEG (Qeeg) data for Group 1 (tPBM), and for Group 2 (wbPBM) separately, are presented in Figure 3A,B. For Group 1, only the P300 T (sec) showed significant improvement (decrease in time). For Group 2, there were significant improvements in P300 V (mV); Flanker T (sec); and Flanker V (mW) (p < 0.05 and beyond).
3.4.1 P300—Time and voltage
The functional EEG assessments demonstrated a significant reduction in P300T following tPBM (0.307 ± 0.03 to 0.277 ± 0.01 s, n = 7, p = 0.028) and wbPBM (0.3 ± 0.03 to 0.29 ± 0.04 s, n = 7, p = 0.583) (Figure 3A,B,C). While the tPBM group noted statistically significant reduction in P300T, the wbPBM group noted a similar reduction, but this was not statistically significant. Concurrently, an increase in P300V was noted following tPBM (12.34 ± 5 to 16.43 ± 4.2 mV, n = 7, p = 0.125), and wbPBM (9.34 ± 6.13 to 15.71 ± 4.3 mV, n = 7, p = 0.046) (Figure 3A,B,D). In contrast to the P300T, the P300V noted a consistent increase in the wbPBM group that was statistically significant, while the tPBM increase was not significant. These assessments did not demonstrate any statistically significant difference in either P300T or P300V among gender (Female −0.017 ± 0.37 s and 5.34 ± 3.4 mV, n = 10 vs. Male −0.42 ± 0.05 s and 4 ± 3.3 mV, n = 4, p = 0.37 and 0.52, respectively), or age (< 65 years −0.02 ± 0.03 s and 5.24 ± 2.97 mV, n = 9 vs. >65 years −20.03 ± 0.05 s and 4.4 ± 4.12 mV, n = 5, p = 0.71 and 0.71, respectively).
3.4.2 Flanker—Time and voltage
The functional EEG assessments demonstrated a reduction in FlankerT following tPBM (0.52 ± 0.08 to 0.45 ± 0.08 s, n = 7, p = 0.1), and wbPBM (0.56 ± 0.09 to 0.47 ± 0.07 s, n = 7, p = 0.045) (Figure 3A,B,E). Both the tPBM and wbPBM groups observed a reduction in FlankerT, but it was only statistically significant in the latter group. An increase in FlankerV was noted following tPBM (13.14 ± 9.7 to 20.87 ± 12.2 mV, n = 7, p = 0.215), and wbPBM (7.61 ± 2.89 to 13.1 ± 3.53 mV, n = 7, p = 0.008) (Figure 3A,B,F). Thus, the FlankerV also demonstrated a similar trend, with only the wbPBM group noting a statistically significant increase. No statistically significant differences in either P300T or P300V among gender (Female −0.099 ± 0.47 s and 6.37 ± 6.65 mV, n = 10 vs. Male −0.052 ± 0.06 s and 6.4 ± 5.14 mV, n = 4, p = 0.22 and 0.99 paired T-test respectively), age (< 65 years −0.10 ± 0.05 s and 6.67 ± 7.02 mV, n = 9 vs. >65 years −0.07 ± 0.65 s and 5.86 ± 4.52 mV, n = 5, p = 0.41 and 0.80 paired T-test respectively).
4 DISCUSSION
The COVID-19 pandemic has had far-reaching implications on many aspects of global society, including public health, access to medical care, the global economy, policy, and politics. It has also demonstrated our tremendous capabilities in biomedicine, such as vaccination, monoclonal antibodies, immunomodulators, and antiviral agents. While it is anticipated that SARS-CoV-2 infections will become less prevalent and less severe, their long-term impact on the global health of approximately 200 million known cases is yet to be fully confronted [63–65]. One of the most serious of these is emerging reports on cognitive problems among those with pre-existing neurological conditions, such as Parkinson’s disease, Alzheimer’s disease, and mild cognitive impairment. Cognitive impairment has been recognized as a part of chronic illness that results in immobility and social isolation.
Transcranial PBM treatments with near-infrared light have been noted to penetrate deeper anatomical sites effectively [66–68]. The use of PBM therapy has shown significant therapeutic efficacy in acute and chronic brain conditions such as Parkinson’s disease, Alzheimer’s disease, dementia, mild cognitive impairment, traumatic brain injury, post-traumatic stress disorder, Gulf War illness, and depression, among many others [48, 69–83]. Besides these disease models with compromised neurocognition, there have been clear demonstrations of the ability of PBM to improve memory, attention, emotion, and executive functions [84–88]. An improvement in local circulation and modulation of Cytochrome C oxidase activity in the discrete parts of the brain, especially the prefrontal cortex, following PBM treatments has been proposed as a potential therapeutic mechanism [89–91]. There has been significant progress in our understanding of the molecular mechanisms of PBM in three discrete cellular sites. The first PBM mechanism described involves intracellular mitochondrial cytochrome C oxidase that transiently increases adenosine triphosphate (ATP) and reactive oxygen species (ROS) generation following photoabsorption [92, 93]. The second site of PBM interactions has been the description of specific photoresponsive cell membrane receptors and transporters, such as non-visual opsins, and TRPV-1, among others [94–98]. Finally, an extracellular PBM mechanism involving direct activation of latent TGF-β1 involving a redox-mediated conformational change has been described [99]. The role of these specific pathways to enable improved neuroplasticity and reduced neuroinflammation has contributed to improved cognition [100–102].
In designing this study, our major hypothesis was that transcranial treatments with a 1070 nm PBM device would be more effective in alleviating COVID brain fog than whole-body PBM treatments with a 660 and 850 nm bed [70]. In contrast to our expectations, both devices performed equivalently and the bed in fact appears to have more, albeit statistically insignificant, improvement. This could be potentially attributed to the increased cumulative dose based on the significant differences in scalp versus total body surface area. The clinical safety of these transcranial PBM treatments has been previously demonstrated [16]. A recent human clinical study demonstrated the utility of a whole-body, transdermal PBM treatment in COVID patients [18]. This motivated us to compare the two PBM delivery modalities in this pilot study focusing on the potential mitigation of multiple long COVID symptoms. It has been well documented that assessment of outcomes is usually a combination of musculoskeletal and neurocognitive functions [103–105]. In fact, a recent study noted several similarities between chronic fatigue syndrome and post-COVID-19 sequelae on physical fatigue, poor sleep quality, increased anxiety, depressive symptoms, and perturbation of a wide range of attentional and visuospatial cognitive domains [106]. This is, to our knowledge, the first human clinical report on utilizing a whole-body PBM treatment to target a central neural ailment, although similar approaches have been successfully employed in animal models [49]. The equipoise noted with both PBM treatment approaches, as noted in prior studies as well indicates that the evoked therapeutic biological responses indicate a prominent systemic component. The nature of the mechanism mediating these responses remains to be investigated.
The cognitive impairment, brain fog, in patients who have recovered from acute SARS-CoV-2 is typically less pronounced than in neurodegenerative conditions, such as Alzheimer’s disease [41, 107]. Assessments that have been developed for these conditions are less useful for assessing brain fog, as patients typically score in the normal range for MMSE, MoCA, and trail-making tests. Some traditional methods of assessing cognitive impairment developed for evaluating patients with Alzheimer’s disease or Lewy-body dementia, including the Montreal Cognitive Assessment (MoCA) and Trail making tests A and B (TRA, TRB), were not useful in evaluating subjects with milder, but still quite troublesome, brain fog [108, 109]. We observed improvements in the MoCA, TrA and B, and the DSST scores after PBM treatments with each of the treatment modalities.
The qEEG has been used in a clinical setting to assess evoked potential differences between patients with lower versus higher cardiac risk. Further, a prior study has demonstrated the utility of qEEG in assessing the utility of PBM in mitigating dementia [110]. There was no difference in effect on delay times or voltages between participants treated with wbPBM or tPBM, neither after the first treatment nor cumulatively over the full course of treatments. Each method significantly decreased the Flanker (visual ERP) response time and increased the Flanker voltage and P300 (auditory ERP) voltage, implying an improvement in physical brain processing speed and power. Only one of the 14 participants treated with tPBM failed to improve on the Flanker or P300 voltages, but that participant did shorten the Flanker response time; he/she had been treated with. The use of qEEG allowed assessment of brain function, specifically neurological responses to auditory (P300) and visual (Flanker) evoked potentials. These responses included both processing speed (delay time) and processing power (voltage). We postulated that measuring brain processing speed and power by using auditory and visual evoked potentials could be more sensitive than cognitive tests and could be practically employed in a clinic setting for diagnostic purposes as well as evaluation of interventional efficacy.
We would like to draw attention to the utility of the recently described photon fluence and Einstein PBM dose concept. This dose equivalence includes the individual wavelength photon energy of the tPBM with the helmet (1070 nm 1.2 eV) and the wbPBM with the bed (red 660 nm 1.9 eV and infrared 850 nm 1.5 eV). This is particularly relevant for two reasons. First, accounting for the discrete wavelengths accounts for delivering precise amounts of low dose energy that enable PBM efficacy. Moreover, this approach provides a rationalized comparative assessment of the single versus dual combined wavelength, as evident with the wbPBM (7.9 Einstein) versus tPBM (6.0 Einstein). A major advantage of this approach is its ability to enable harmonized dose interpretation and communication that can be universally implemented with accessible PBM wavelength devices that may otherwise be globally restrictive.
This study is limited by both the number of subjects and the lack of a placebo arm. Further study with a larger population as well as subject masking is planned. Repeated neurophysiological testing (6 tests over 4 weeks) with MoCA, DSST, and trail-making tests could conceivably improve performance as a result of gaining familiarity with the test and practice; however, no papers have been published for a learning effect on these tests. Such an effect is less likely with qEEG parameters, as there is no evidence that processing speeds or power can be influenced by repeated practice, and placebo effects are less likely to persist over a full course of treatment [74, 111]. In addition, further examination of current data is planned to determine if biomarkers provided by qEEG can be used to predict, early in the clinical treatment course, which patients would most likely benefit from PBM treatment. Other treatment modalities could likewise use these biomarkers to determine the possible treatment efficacy of those other modalities in each individual patient.
In summary, PBM delivered by either whole body or transcranial treatment was effective in improving parameters of brain performance in subjects with at least 5 months duration of cognitive impairment after infection with COVID-19. The choice of wbPBM in an office setting versus at-home tPBM, which has shown efficacy with TBI and dementia, should be explored further. It is conceivable that a synergistic combination of the in-clinic and at-home PBM treatments, along with an emphasis on nutrition and exercise, could ideally address access, costs, and compliance issues, especially with chronic diseases.
ACKNOWLEDGMENTS
We would like to acknowledge Shepherd University and the West Virginia Governor Jim Justice’s office for providing funding for this study. We also thank Mary Hendrix, Sharon Mailey, Donald Patthoff, and the Foundation for Photobiomodulation Research for their vision, encouragement and support for this work.
CONFLICT OF INTEREST STATEMENT
The authors declare they have no conflicts of interest with this study.